Thermodynamics – Complete Guide For Class 11 Chemistry Chapter 5
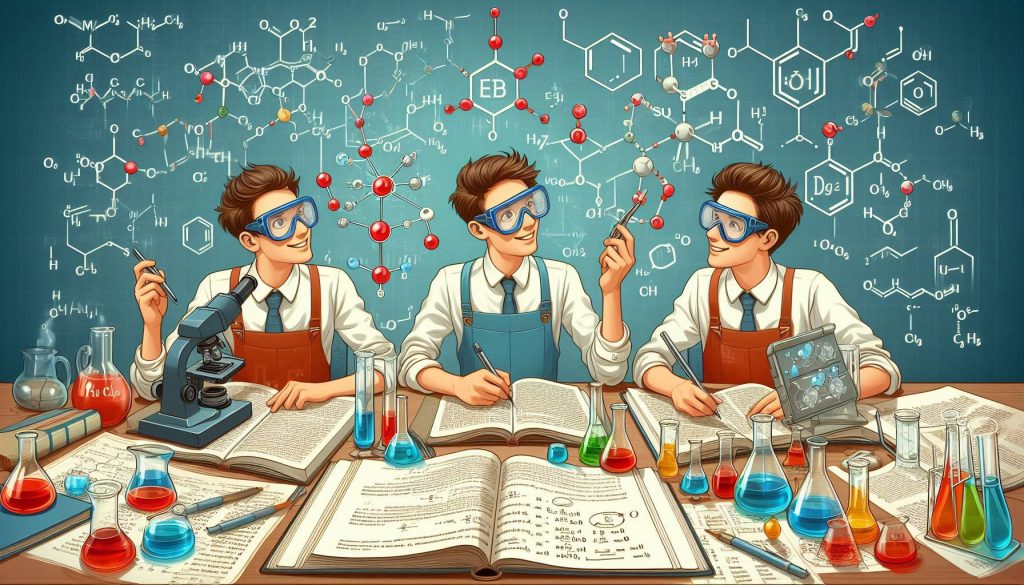
Welcome to iPrep, your Learning Super App. Our learning resources for Chapter 5, “Thermodynamics,” in Class 11 Chemistry are meticulously designed to ensure students gain a comprehensive understanding of this essential topic. These resources include detailed notes on the fundamental principles of thermodynamics, such as the first and second laws, which explain energy conservation and entropy. They also cover various thermodynamic processes, like isothermal and adiabatic changes, and concepts such as enthalpy, Gibbs free energy, and spontaneity of reactions.
Furthermore, we provide illustrative examples, diagrams, and problem-solving strategies to help students apply these concepts to real-world biological and chemical systems. With these tools, students are well-equipped to excel in their studies and grasp the intricate relationships between energy and matter in living organisms.
This chapter examines how living organisms manage energy and matter to maintain order, grow, and perform various functions. Key topics include the laws of thermodynamics, which explain how energy is conserved and how entropy, or disorder, changes in a system. Understanding these principles is crucial for comprehending how metabolic processes work, how cells harness energy, and how life sustains itself through complex biochemical reactions.
Thermodynamics: A Comprehensive Guide
Thermodynamics is a branch of physics that deals with the relationship between heat and other forms of energy. It provides a framework for understanding the behavior of matter and energy at a macroscopic level. In this blog post, we will explore the fundamental concepts of thermodynamics, including systems, surroundings, state functions, internal energy, work, heat, and the First Law of Thermodynamics.
Systems and Surroundings
In thermodynamics, a system is the part of the universe that we are interested in studying. The rest of the universe is considered the surroundings. Together, the system and its surroundings make up the universe.
There are three main types of systems:
- Open system: Exchanges both matter and energy with the surroundings.
- Closed system: Exchanges only energy with the surroundings.
- Isolated system: Exchanges neither matter nor energy with the surroundings.
State Functions and Internal Energy
The state of a thermodynamic system is described by its measurable properties, such as pressure (p), volume (V), temperature (T), and amount (n). Variables like p, V, and T are called state functions because their values depend only on the current state of the system and not on how it reached that state.
The internal energy (U) of a system is the total of all the possible kinds of energy within the system. It is a state function and depends only on the state of the system, not on how it was reached.
Work, Heat, and the First Law of Thermodynamics
Work is the transfer of energy between a system and its surroundings due to a force acting over a distance. Heat is the transfer of energy between a system and its surroundings due to a temperature difference.
The First Law of Thermodynamics states that the total energy of an isolated system remains constant. In other words, energy cannot be created or destroyed, only transferred or transformed from one form to another. Mathematically, the First Law is expressed as:
ΔU = Q – W
Where:
- ΔU is the change in the internal energy of the system
- Q is the heat transferred to the system
- W is the work done by the system
Enthalpy and Enthalpy Changes
Enthalpy (H) is a thermodynamic property that represents the total heat content of a system. It is defined as:
H = U + PV
Where:
- U is the internal energy
- P is the pressure
- V is the volume
The enthalpy change (ΔH) of a reaction is the heat absorbed or released during the reaction at constant pressure. It is a measure of the energy difference between the reactants and products.
Spontaneous Processes and Entropy
A spontaneous process is a process that occurs naturally without external intervention. The entropy (S) of a system is a measure of its disorder or randomness. The Second Law of Thermodynamics states that the total entropy of the universe always increases or remains constant for a spontaneous process.
Thermodynamic Processes
Thermodynamic processes describe the transition of a system from one state to another. Here are the key types of thermodynamic processes:
- Isothermal Process: A process that occurs at a constant temperature (dT=0).
- Adiabatic Process: A process in which no heat is transferred to or from the system (dq=0).
- Isobaric Process: A process that occurs at a constant pressure (dp=0).
- Isochoric Process: A process that occurs at a constant volume (dV=0).
- Cyclic Process: A process that returns a system to its initial state, meaning changes in internal energy (dE=0) and enthalpy (dH=0) are zero.
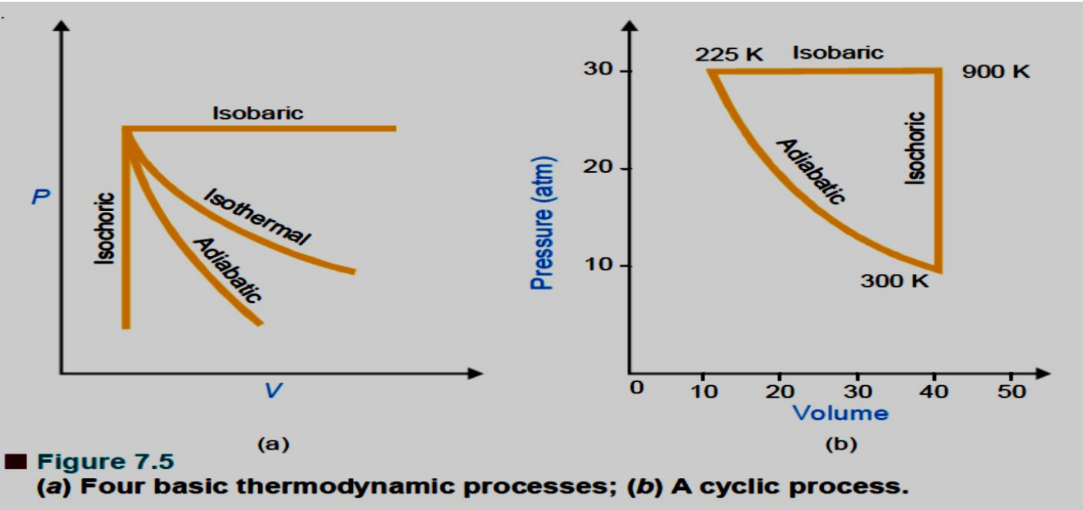
Reversible Processes
A reversible process is an ideal process that can be reversed without leaving any change in the surroundings. The key characteristics of reversible processes are:
Definition of a Reversible Process
- Reversible Process: A process whose direction can be reversed by inducing infinitesimal changes to some property of the system via its surroundings, while not increasing entropy.
Alternatively, a reversible process can be defined as:
- Reversible Process: A process that can be reversed without leaving any change in the surroundings.
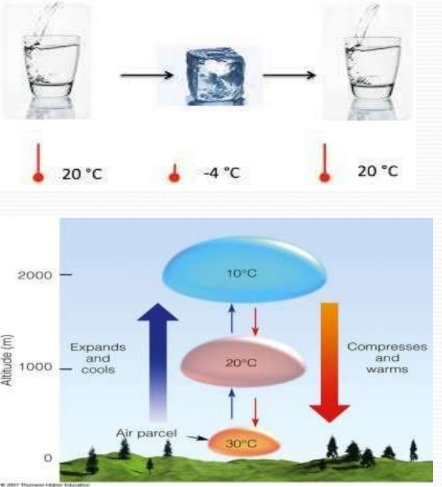
Characteristics of a Reversible Process
- A reversible process passes through a continuous series of equilibrium states.
- It can be stopped at any stage and reversed so that the system and surroundings are exactly restored to their initial states.
For example, consider the expansion of a gas in a piston as shown in the figure. The gas expands by removing infinitesimal weights slowly from the piston one by one. This process passes through equilibrium states and tends towards a reversible process. The gas can be brought back by compression after putting weights back on the piston.
Idealized Examples of Reversible Processes
Some processes that can be idealized as reversible processes include:
- Frictionless relative motion
- Expansion and compression of a spring
- Frictionless adiabatic expansion or compression of a fluid
- Isothermal expansion or compression
- Elastic stretching of a solid
- Electrolysis process
A reversible process produces the maximum work in engines and requires the minimum work in devices such as heat pumps.
Irreversible Processes
An irreversible process is a process that cannot be reversed without leaving a change in the surroundings. Unlike reversible processes, irreversible processes involve a series of non-equilibrium states.
Definition of an Irreversible Process
- Irreversible Process: A process that is not reversible. In an irreversible process, the system passes through a series of non-equilibrium states.
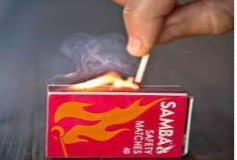
Characteristics of an Irreversible Process
- It is difficult to locate properties on a property diagram as they do not have a unique value.
- When an irreversible process is made to proceed in the backward direction, it does not reach its original state.
- The system reaches a new state.
- Irreversible processes are usually represented by dotted lines on diagrams.
Factors Causing Irreversibility
The factors that cause a process to be irreversible include:
- Friction
- Free Expansion
- Mixing of two gases
- Heat transfer between finite temperature differences
- Electric resistance
- Inelastic deformation
- Chemical reactions
The presence of any of these effects makes the process irreversible.
Examples of Irreversible Processes
Examples of irreversible processes include:
- Relative motion with friction
- Combustion
- Diffusion of gases: mixing of dissimilar gases
- Chemical reactions
- Free expansion and throttling processes
- Plastic deformation
- Electricity flows through a resistance
Some Important Facts on Entropy
Entropy is a fundamental concept in thermodynamics that relates to the level of disorder in a system. Here are some crucial points about entropy:
- Maximum Disorder in Equilibrium State: An equilibrium state has the maximum disorder; hence, the entropy in the equilibrium state is the highest.
- Temperature and Entropy: Entropy increases when the temperature is increased.
- Creation and Conservation of Entropy: Entropy can be created but never destroyed. Therefore, the entropy of the system and its surroundings can never be reduced.
- Mechanical Energy and Entropy: The conversion of mechanical energy into heat energy increases entropy, and vice versa.
- Relation to Absolute Temperature: Entropy is related to absolute temperature only.
Heat
Heat is another critical concept in thermodynamics, referring to the energy transfer between systems or surroundings due to temperature differences.
- Internal Energy Change through Heat Transfer: We can change the internal energy of a system by transferring heat from the surroundings to the system or vice-versa without any expenditure of work. This exchange of energy, due to a temperature difference, is called heat, denoted as qqq.
For example, consider transferring heat to water in a copper container. If the temperature of the water is TA and the container is in a large heat reservoir at temperature TB, the heat absorbed by the system (water) q can be measured in terms of the temperature difference, TB−TA. In this scenario, the change in internal energy ΔU=q\Delta U = qΔU=q when no work is done at constant volume.
- Sign Convention: The heat q is positive when heat is transferred from the surroundings to the system and negative when heat is transferred from the system to the surroundings.
First Law of Thermodynamics
The first law of thermodynamics is a version of the law of conservation of energy. It states that the total energy of an isolated system remains constant, though it may change from one form to another.
Mathematical Statement of the First Law
The first law can be expressed mathematically as:
ΔE=q−w
Where:
- ΔU = Change in internal energy of the system
- qqq = Heat absorbed by the system (positive if heat is absorbed, negative if heat is released)
- www = Work done on the system (positive if work is done on the system, negative if work is done by the system)
The change in internal energy (ΔU) depends on the heat added to the system and the work done on it.
Thus, the first law may also be stated as: the net energy change of a closed system is equal to the heat transferred to the system minus the work done by the system.
Special Cases of the First Law of Thermodynamics
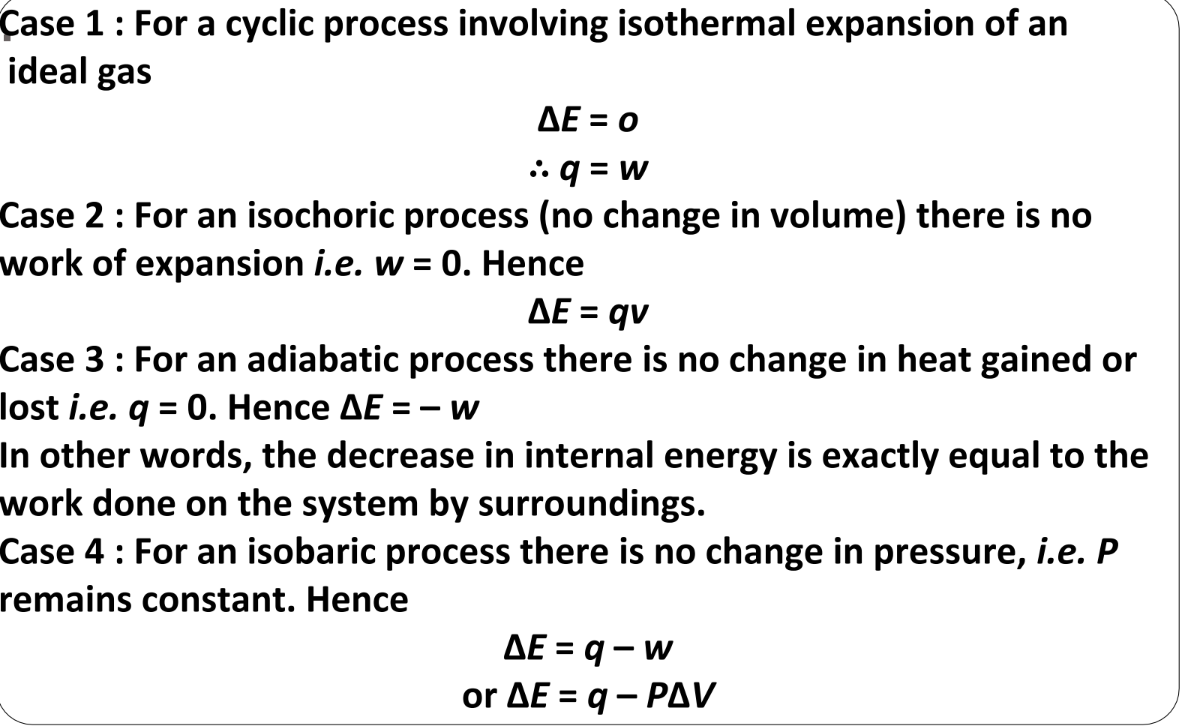
Explanation of the First Law of Thermodynamics
Let’s consider a general case where a change of state is brought about by both doing work and transferring heat. The change in internal energy for this case is:
ΔU=q+w
For a given change in state, qqq and www can vary depending on how the change is carried out. However, q+w=ΔUq + w will depend only on the initial and final state, and it will be independent of how the change is carried out. If there is no transfer of energy as heat or as work (isolated system), i.e., if w=0 and q=0, then ΔU=0.
The equation ΔU=q+w is a mathematical statement of the first law of thermodynamics, which states:
- Energy Conservation: The energy of an isolated system is constant. This law is commonly stated as the law of conservation of energy, i.e., energy can neither be created nor destroyed.
Example Scenarios
- No Heat Absorbed, Work Done on System:
- ΔU=wad, wall is adiabatic.
- No Work Done, Heat Removed:
- ΔU=−q, thermally conducting walls.
- Work Done by System, Heat Supplied:
- ΔU=q−w, closed system.
Applications of the First Law of Thermodynamics
Work – Pressure-Volume Work
To understand pressure-volume work, consider a cylinder containing one mole of an ideal gas fitted with a frictionless piston. The total volume of the gas is V and the pressure of the gas inside is p. If the external pressure pex is greater than p, the piston is moved inward until the pressure inside equals pex. If this change is achieved in a single step and the final volume is Vf, the work done on the gas can be calculated.
Work Done by an Expanding Gas at Constant Pressure
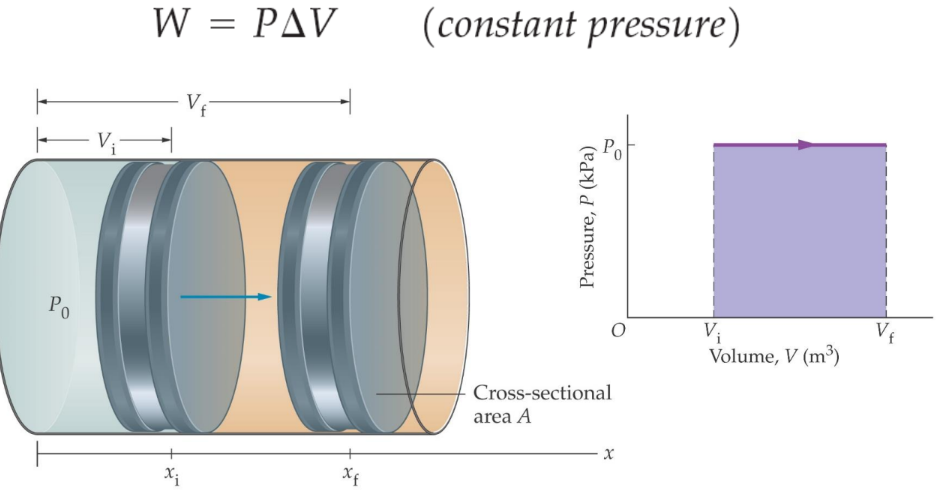
During this compression, suppose the piston moves a distance lll and the cross-sectional area of the piston is AAA, then the volume change is l×A=ΔV=(Vf−Vi)
The pressure can be defined as:
Pressure=Force/Area
Thus, the force on the piston is pex⋅A. If w is the work done on the system by the movement of the piston, then:
W=Force×Displacement=pex×A×l
The negative sign indicates that in the case of compression, work is done on the system. Here, (Vf−Vi) will be negative, and multiplying two negative numbers will yield a positive sign for the work done.
Non-Constant Pressure
If the pressure is not constant at every stage of compression but changes in several finite steps, the work done on the gas will be summed over all the steps and will be equal to:
−w=−∑ΔpΔV
In the case where the pressure changes infinitesimally during the process and is always slightly greater than the pressure of the gas, at each stage of compression, the volume decreases by an infinitesimal amount of dV. The work done on the gas can then be calculated using an integral form:
−w=∫ViVfpex dV
Reversible Processes
A reversible process or change is one where the change is brought out so that the process could, at any moment, be reversed by an infinitesimal change. A reversible process proceeds infinitely slowly through a series of equilibrium states, such that the system and surroundings are always in near equilibrium. Processes that are not reversible are known as irreversible processes.
Free Expansion
The expansion of a gas in a vacuum (pex=0) is called free expansion. No work is done during the free expansion of an ideal gas, regardless of whether the process is reversible or irreversible. Here, w=−pexΔV. Thus, the internal energy change ΔU=q−pexΔV simplifies to ΔU=qv, indicating that heat is supplied at constant volume.
Isothermal and Free Expansion of an Ideal Gas
For the isothermal (constant temperature) expansion of an ideal gas into a vacuum:
- Irreversible Change: w=0 since pex=0, and since q=0 experimentally determined by Joule, ΔU=0.
- Reversible Change: q=−w=nRTln(Vi/Vf)
- For Adiabatic Change: When q=0, ΔU=Wad
Enthalpy (H)
Enthalpy is a thermodynamic property that represents the total heat content of a system. It is an extensive property, meaning it depends on the amount of substance present in the system. The change in enthalpy (ΔH) during a process is equal to the heat absorbed or released at constant pressure.
Extensive and Intensive Properties
- Extensive Properties: These properties depend on the amount of matter in a system. Examples include mass, volume, and enthalpy.
- Intensive Properties: These properties do not depend on the amount of matter in a system. Examples include temperature, pressure, and density.
Heat Capacity
Heat capacity is the amount of heat required to change the temperature of a substance by one degree Celsius. It is an extensive property and can be defined as:
C=qΔTwhere q is the amount of heat added and ΔT is the temperature change.
- Specific Heat Capacity: It is the heat capacity per unit mass of a substance.
- Molar Heat Capacity: It is the heat capacity per mole of a substance.
Calorimetry
Calorimetry is the science of measuring the heat of chemical reactions or physical changes. A calorimeter is a device used to measure the heat absorbed or released during these processes.
Relationship Between ΔH and ΔE
The change in enthalpy (ΔH) and the change in internal energy (ΔE) are related by the equation:
ΔH=ΔE+Δ(PV)
Where Δ(PV) is the change in the product of pressure and volume. For reactions occurring at constant pressure, the enthalpy change is equal to the heat absorbed or released.
Example: Enthalpy Change
Consider a reaction occurring in a closed system at constant pressure. If the reaction absorbs 150 J of heat and performs 50 J of work, the change in enthalpy (ΔH) can be calculated as:
ΔH=q+w=150J−50J=100J
Enthalpies of Different Types of Reactions
- Enthalpy of Formation (ΔHf∘): The enthalpy changes when one mole of a compound is formed from its elements in their standard states.
- Enthalpy of Combustion (ΔHc∘): The enthalpy changes when one mole of a substance is completely burned in oxygen.
- Enthalpy of Fusion (ΔHfus): The enthalpy changes when one mole of a solid substance melts to become a liquid.
- Enthalpy of Vaporization (ΔHvap): The enthalpy changes when one mole of a liquid evaporates to become a gas.
Laws of Thermochemistry
- Lavoisier and Laplace Law: The energy change for a chemical reaction is equal in magnitude but opposite in sign to the energy change for the reverse reaction.
- Hess’s Law: The enthalpy change of a chemical reaction is the same regardless of the pathway by which the reaction occurs, as long as the initial and final conditions are the same.
Bond Enthalpies
Bond enthalpy, also known as bond dissociation energy, is the energy required to break one mole of a particular bond in a gaseous molecule. It is an average value because it varies depending on the molecular environment.
Spontaneity
A process is spontaneous if it occurs without the need for continuous external influence. Spontaneity depends on two factors:
- Enthalpy Change (ΔH): Processes that release energy (exothermic reactions) tend to be spontaneous.
- Entropy Change (ΔS): Processes that increase disorder (entropy) tend to be spontaneous.
Gibbs Energy Change and Equilibrium
Gibbs free energy (G) is a thermodynamic potential that predicts the spontaneity of a process at constant pressure and temperature. The change in Gibbs free energy (ΔG) is given by:
ΔG=ΔH−TΔS
- ΔG < 0: The process is spontaneous.
- ΔG = 0: The system is at equilibrium.
- ΔG > 0: The process is non-spontaneous.
Standard Gibbs Energy of Formation
The standard Gibbs energy of formation (ΔGf∘) is the change in Gibbs energy when one mole of a compound is formed from its elements in their standard states.
Conclusion
In conclusion, Class 11 Chemistry Chapter 5: Thermodynamics offers students a deep insight into the fundamental principles governing energy and matter in physical and biological systems. By mastering key concepts like the laws of thermodynamics, enthalpy, entropy, and Gibbs free energy, students can develop a robust understanding of how these ideas apply to real-world scenarios.
Through our detailed resources on the iPrep Learning Super App, students can confidently tackle the complexities of thermodynamics, ensuring they are well-prepared for both their exams and future studies. Stay committed to exploring the vast world of thermodynamics, as it forms the backbone of many important scientific fields. Keep learning with iPrep and excel in your journey through CBSE Class 11 Chemistry Chapter 5: Thermodynamics.
Practice questions on Chapter 5 - Thermodynamics
Get your free Chapter 5 - Thermodynamics practice quiz of 20+ questions & detailed solutions
Practice Now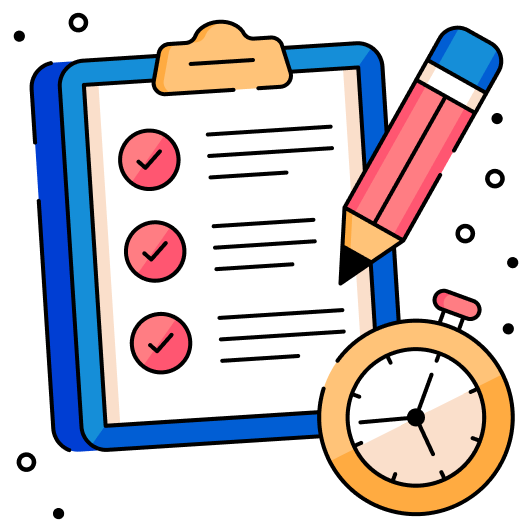